Navigating the terahertz gap – physicsworld.com
The terahertz range has been barely exploited compared to the rest of the electromagnetic spectrum. But as Sidney Perkowitz argues, our ability to detect radiation at these wavelengths has proved vital in many areas of astronomy and cosmology
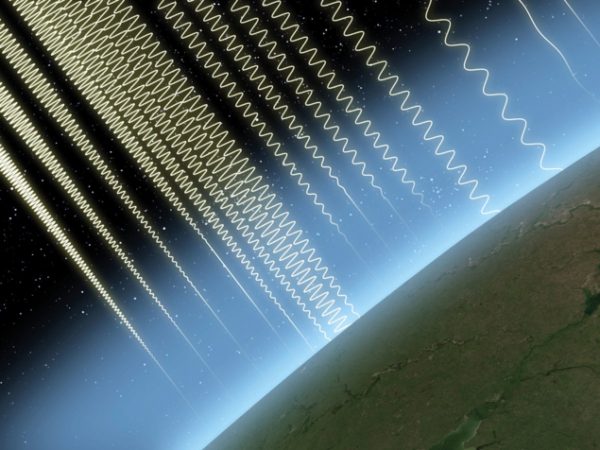
Look carefully at one of those classroom posters that shows the sweep of the electromagnetic spectrum, from gamma rays to radio waves, and you’ll find a small patch squeezed in between the infrared and microwave. Called the far-infrared, submillimetre or terahertz region, it is nominally defined as spanning wavelengths from 30 μm (10 THz) in the mid-infrared, to 1–3 mm (0.1–0.3 THz) in the microwave domain. Although hardly prominent in these educational posters, this region is a rich research area for physics on and beyond the Earth.
While there are microwave and infrared sources that can produce thousands of watts of power at those frequencies, there is a lack of sources that work well across the terahertz range, which is why it is often referred to as the “terahertz gap”. Hot blackbodies only emit microwatts at terahertz frequencies, whereas microwave technology is not easily pushed below millimetre wavelengths, so standard spectroscopic methods do not apply. Yet the gap is well worth exploring. It is the right range to probe electronic, lattice and quantum properties in condensed matter, and to examine massive molecules. It supports applications in biomedicine, in security systems and in the study of artworks. Outside the lab, terahertz radiation is relevant to the cosmic microwave background (CMB) and other astrophysical phenomena, and is helping in the search for the origins of life in space.
Making do with microwatts and milliwatts
Fortunately, when scientists see a gap in understanding, they dive right in. Since the 1960s researchers have found ways to obtain high-quality terahertz spectra by using a Fourier-transform spectrometer. In this device, a hot source sends microwatts of terahertz power to a solid, liquid or gaseous sample where it is reflected or transmitted through. Next, the beam is split into two and each part is reflected from a mirror. The beams are then recombined, and a detector measures the resulting interference signal as one mirror moves relative to the other. Fourier analysis of this interferogram yields the frequency spectrum specific to the sample with a higher signal-to-noise ratio than in a conventional spectrometer.
Another common method of creating a terahertz source – which provides greater power – is by optically pumping the vibrational states of a molecular organic medium, such as methanol (CH3OH), with a CO2 laser. This excites molecular rotational sub-levels that emit discrete terahertz laser lines. Different media supply hundreds of lines at milliwatt powers, which yield precise data over broad ranges.
Since the 1960s and 1970s, in my lab and others, such terahertz Fourier spectrometers and pumped lasers have probed semiconductors and their nanostructures, superconductors, inhomogeneous materials, water in liquid and vapour form, and biomolecules. But more recently, lab research has been enhanced by powerful new terahertz sources based on semiconductor technology (see June 2016), and on synchrotrons and free electron lasers (see box, below). Indeed, according to a 2014 bibliometric study by Roger Lewis of the University of Wollongong in Australia, the number of papers published containing “terahertz” in the abstract, title or keywords grew exponentially between 1975 and 2013 (J. Phys. D: Appl. Phys. 47 374001).
Power for the terahertz gap
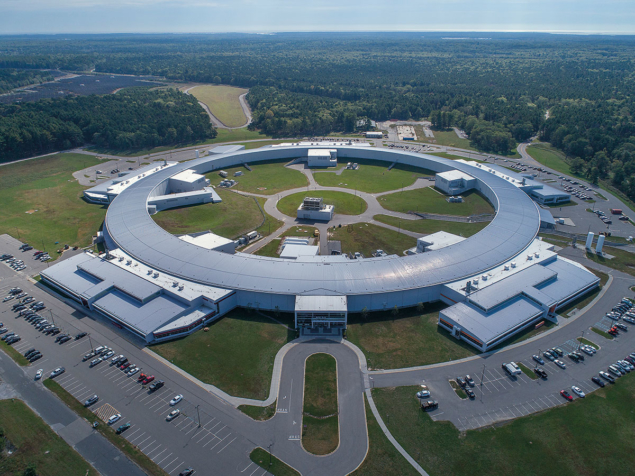
Spacecraft-based astrophysical and cosmological research at terahertz frequencies – such as measuring the fluctuations in the cosmic microwave background – is made possible thanks to sensitive detectors that have been cryogenically cooled to reduce noise, as in the COBE and Planck projects. Sensitive detectors are also important for terahertz Fourier-transform spectroscopy in the lab that uses microwatts from a hot source.
Another approach for terahertz spectroscopy is to develop more powerful sources. Optically pumped lasers (see main text) generate milliwatts, which is ample for many uses, but they require a CO2 laser and, unlike a blackbody, do not offer continuous frequency coverage. Other powerful sources have different limitations. Quantum cascade lasers (QCLs, see June 2016 pp28–31) are semiconductor nanostructures that produce higher powers, but at fixed frequencies and under cryogenic cooling. One recent paper (L H Li et al. 2017 Electronics Letters 53 799) describes a QCL that emits 1.8 W and 2.4 W at 4.4 THz, cooled to 77 K and 10 K, respectively.
High powers for demanding applications such as terahertz microscopy are also available at central facilities. One type of source generates powerful terahertz waves in a free-electron laser, where a beam of relativistic electrons moves past an arrangement of magnets with alternating poles. This gives the electrons a transverse wiggling motion, which produces monochromatic photons whose frequency can be tuned by changing the electron velocity or the magnetic field, and that are made coherent by confinement in a cavity. The free-electron laser at the University of California, Santa Barbara, for example, generates kilowatts from 0.1 THz to 4.8 THz. Another unit at the Budker Institute of Nuclear Physics in Novosibirsk, Russia, operates from 1.2 to 8.2 THz.
High-power terahertz radiation is also generated by electrons circulating in a synchrotron storage ring. The National Synchrotron Light Source at the Brookhaven National Laboratory in New York maintains a terahertz beamline that provides 100 mW of broadband power at frequencies above 0.15 THz, covering the terahertz range and beyond and acting as a source for a Fourier-transform spectrometer. A beamline at another synchrotron, the Canadian Light Source at the University of Saskatchewan in Saskatoon, also covers the terahertz range at high brightness as a source for Fourier-transform spectrometry and terahertz microscopy.
With judicious choice of detectors, sources or both, scientists have found footholds in the terahertz gap and perform research of the highest quality. But the ideal terahertz source – producing milliwatts or more, tunable over the entire range, compact and operating at room temperature – remains elusive. This is a major stumbling block to carrying out applications in security systems and biomedicine.
Terahertz physics takes to the skies
Back in 1964, terahertz physics also received a powerful push out of the lab and into the universe. That was when Arno Penzias and Robert Wilson, working with an antenna at Bell Labs designed for satellite communications, unexpectedly found a constant signal at the microwave wavelength of 7.35 cm that seemed evenly distributed across the heavens.
The definitive measurement of this unknown radiation was made aboard NASA’s Cosmic Background Explorer (COBE) satellite, which launched in 1989. Using a Fourier-transform spectrometer, COBE’s result was in near-perfect agreement with the emission curve of a blackbody at 2.725 ± 0.002 K. With peak intensity at 1.07 mm, this spectrum spans the terahertz range (figure 1), and Penzias and Wilson had picked up the tail end of the curve. Separately, COBE also compared millimetre-wave radiation from different sky directions and found that the CMB is slightly anisotropic, representing temperature fluctuations of 1 part in 105 (see February 2020).
1 Close curve
The cosmic microwave background data from COBE, which shows a remarkably close fit to the emission curve of a blackbody at 2.7 K, actually enters the terahertz range.
The blackbody data matched a 1965 prediction by the cosmologists Robert Dicke, Philip Peebles and colleagues that as the universe cooled after the Big Bang, it would be filled with residual blackbody radiation at ~3 K. This agreement provided strong support for the Big Bang theory and the results gave deep insights into the history of the universe. Indeed, when George Smoot and John Mather received the 2006 Nobel Prize for Physics for their work on COBE, the Nobel Committee noted that COBE can be “regarded as the starting point for cosmology as a precision science”.
The CMB temperature fluctuations are also significant; they represent density variations in the hydrogen making up the universe 380,000 years after the Big Bang. These evolved into today’s cosmic structure, with filaments of galaxies surrounded by enormous voids. After COBE, the fluctuations were studied from space by NASA’s Wilkinson Microwave Anisotropy Probe (WMAP) launched in 2001, and the Planck spacecraft, launched by the European Space Agency in 2009. Advances in detection methods and space technology improved each subsequent mission. Planck sensed weak signals with heat detectors cooled to 0.1 K, giving attowatt noise levels, and covered the widest frequency range at nine values from 0.03 THz (10 mm) to 0.857 THz (0.35 mm). The spacecraft measured cosmic temperature differences of 5 μK or less at angular resolutions down to 4 arcmin, compared to 7° for COBE and 0.5° for WMAP (figure 2).
2 All in the detail
Successively higher resolution of temperature variations in the early universe as seen in the terahertz range by the COBE, WMAP and Planck spacecraft.
The terahertz data from Planck was analysed with the so-called lambda cold dark matter model (ΛCDM), the cosmological “standard model”. ΛCDM assumes that physics, including general relativity, is the same throughout the universe; that the universe was initially hot and dense and has always been expanding; and that it includes dark energy, dark matter, ordinary matter, photons and neutrinos. In 2018 the final results from Plank showed that the universe is 13.8 billion years old; it contains 31.5% matter (4.9% normal matter and 26.6% dark matter) and 68.5% dark energy; it most probably contains only three species of neutrinos, whose masses sum to less than 0.12 eV; and it is expanding with a Hubble constant H0 of 67.4 km/s/Mpc.
These results provide our most accurate and comprehensive picture of the universe to date and terahertz detection technology played an important role. However, with an uncertainty of only 1%, the Planck value of H0 is at variance with the value 73 km/s/Mpc derived from other supposedly reliable astrophysical data – a difference that perhaps points to new physics.
Seeing a black hole with millimetre waves
Another terahertz astrophysical project required far higher resolution than Planck attained. In April 2019 the international Event Horizon Telescope (EHT) collaboration presented the first ever image of a black hole – the supermassive black hole at the centre of the elliptical galaxy M87, 55 million light-years away.
The aim had been to study the region near the event horizon by observing the black hole’s “shadow”, a dark area within the glow emitted by hot accretion material flowing into the black hole. The shadow, caused by the gravitational bending and capture of light near the event horizon, has a diameter about five times the Schwarzschild radius (the radius of the black hole) as predicted by general relativity. It would subtend only a tiny angle of ~40 μarcsec.
Terahertz photons delineate a black hole because they come from deep within its gravitational well. Earlier studies of M87 at wavelengths from 1.3 mm to 7 mm had shown signs of a central 40 μarcsec structure but could not image it. These results did, however, show that the shorter the millimetre wavelength, the more closely the photons represented the actual site of the black hole within the bright region. But no individual radio telescope installation, single-dish or multi-dish, could provide the required angular resolution at millimetre wavelengths.
The answer was for the EHT to link eight separate installations around the Earth, including the Atacama Large Millimeter/submillimeter Array (ALMA) in the Chilean desert, the South Pole Telescope (SPT) in Antarctica, and the IRAM 30-metre telescope in Spain (figure 3). The resulting virtual telescope gave an angular resolution of the order of the 1.3 mm wavelength divided by the Earth’s diameter. After an intricate process of co-ordinating the telescopes and analysing petabytes of data, the composite network produced a striking image at a resolution of 20 μarcsec. It clearly shows the dark shadow within the bright emission region 42 μarcsec across, which itself displays details. Analysis of the data gave a central mass of 6.5 × 109 solar masses, definitively establishing the existence of a supermassive black hole in M87 and supporting the supposition that black holes of this size lie at the centre of galaxies.
3 Worldwide telescope
The system of telescopes in the Event Horizon Telescope array, forming an Earth-sized virtual telescope to achieve ultrahigh angular resolution of the order of 1.3 mm wavelength divided by the Earth’s diameter. Green dots indicate future sites.
Seeking the molecules of life
Besides research in space to explore the origin and development of the universe, terahertz methods can also examine a different set of fundamental questions. How did life begin on Earth? Was it a unique process, meaning we are alone in the universe? Or did it seed life elsewhere?
One possible answer to these big questions is that the complex molecules of life, or their precursors, originated in the interstellar medium, and came to Earth and other planets via meteorites. According to what we know about earthly life, this means finding organic molecules in space that contain carbon along with hydrogen, oxygen and nitrogen. Some of these molecules – including the amino acids necessary to build proteins – have already been found in meteorites that landed on Earth, and now terahertz astronomical spectroscopy is being used to seek such biotic or pre-biotic molecules in space.
The universe seems to support active carbon-based chemical processes – indeed, the first molecule found in space was CH in 1937, and organic molecules still dominate the more than 200 species found since by ultraviolet to centimetre-wavelength spectroscopy. These results mostly come from radio astronomy at frequencies below 2 THz, where transitions between the energy levels associated with molecular rotations provide many identifying spectral features in emission or absorption. This is the same mechanism that in the lab generates terahertz laser lines from compounds like methanol, CH3OH (which has also been found in space).
The known astronomical organic molecules contain up to 13 atoms (excluding the non-biotic fullerenes C60 and C70), a level of complexity associated with biological function. In 2003 the simple amino acid glycine (NH2CH2COOH) was reportedly detected in space, but later measurements have not confirmed this. Other relevant findings are the sugar-related molecule glycolaldehyde (CH2OHCHO), and formamide (NH2CHO), a possible biotic precursor with the appropriate properties to form sugars and amino acids.
The high angular resolution offered by arrays like ALMA aids the search for complexity beyond the straight-chain carbon backbone found in most big organic molecules in space. In 2014 a team under Arnaud Belloche at the Max Planck Institute for Radio Astronomy in Bonn, Germany, used ALMA at 3 mm wavelength to find the first space molecule with a branched carbon chain, iso-propyl cyanide (i-C3H7CN). This feature is characteristic of the amino acids that have been seen in meteorites on Earth. The molecule was observed in the giant star-forming gas cloud Sagittarius B2 in our galaxy, suggesting that active areas in space tend to make complex compounds. Deeper physical and chemical understanding of how molecules form in diverse places, from interstellar and circumstellar regions to protoplanetary discs, will further focus the hunt for biotic molecules.
The enduring enigma of the cosmic cold spot
Lab measurements of complex molecular spectra are essential as well to guide astronomical research and interpret its results. Susanna Widicus Weaver, for example, is a chemist at Emory University who works on improving terahertz Fourier-transform spectroscopy and other methods, for these purposes and to study new areas in interstellar chemistry, such as molecular reactions with ice. These approaches, she wrote in a recent review article (Ann. Rev. Astron. Astrophys. 57 79), are “poised to fill the terahertz gap…offering analytical techniques that rival those used in the microwave and infrared regions of the electromagnetic spectrum”.
Researchers have extended microwave and infrared methods on and off the Earth to make the terahertz gap navigable and carry out innovative studies in both locales. This breadth illustrates the interdisciplinary nature of terahertz science as it explores the beginnings of the universe, the properties of matter in space and on Earth, and the fascinating, still-mysterious intersection where non-living molecules make the leap into life.